The stars tell a story. About ten years ago, thanks to the Hubble space telescope, it became possible to measure many very distant galaxies, looking back through time in the process. Astronomers could look at the colors of those galaxies. The color of a galaxy is an aggregate of the colors of its myriads of stars. When this was done carefully, with astronomers making sure to note “redness” due to dust, and so on, a fascinating pattern emerged.
15
The colors of galaxies got “bluer” early on in their existence, then peaked about 7 billion years ago, when they began getting redder very quickly.
What do these changing colors mean? Galaxy colors reflect the colors of their constituent stars, so we have to talk about star colors. For about 90 percent or more of its life, a star's color tells us how heavy that star is. As we saw in the last chapter, the more massive a star is, the faster it fuses hydrogen. That makes such stars hotter and more luminous. The extra energy they possess causes them to shine with bluer light, just as the filaments of an incandescent bulb do relative to an electric stove heater. Burning off faster also means that bigger stars have short lives.
So, then, if we see “bluish” galaxies, we can conclude that they have plenty of big, heavy stars and that such big stars are being formed at a high rate. If not for the latter, “bluish”
galaxies would be very rare. Therefore, the colors of distant galaxies can tell us the rate of star formation.
In the Hubble images, the changes of galaxy colors with time show us the changing rate of star formation. Stars and galaxies formed early on, and then stars kept forming at a steadily increasing rate during the following 5 billion years. In the past few billion years that rate has plummeted, worse than the 2008 world markets downturn and with no prospect for recovery. The heady days of big stars are over! Our Galaxy and other galaxies in today's Universe form ten to fifty times fewer stars, and most of the stars are small.
This history is good for chemistry and for life. The bumper crop of early, short-lived stars enriched the Universe and the next generation of stars (and planets) with heavy elements. A galaxy with fewer big blue stars is better for chemistry and life too. X-rays and UV light, which large, “blue” stars create in large quantities, are health hazards. In the past 7â8 billion years our Universe has become more and more hospitable, and will continue to evolve in this direction for a long time in the future.
In the meantime, there are at least two reasons why we should be optimistic about the plurality of worlds with separate origins of life in our Galaxy. First is the apparently short time it took life to emerge and take hold on the surface of the young Earth. Second, we now know that the family of hospitable planets is even larger than we thought, with super-Earths being excellent cradles for life.
CHAPTER TWELVE
THE FUTURE OF LIFE
E
arth is our home planet. We call it the cradle of life. These notions are deeply ingrainedâbut wrong. A home is a place we live in, grow up in, and leave; we buy and sell them. A planet is not a home to life. Rather, the planet and the life on it are the same thing. If I were to leave this discussion here, an astute reader might accuse me of making a trivial point, akin to the politician who refers to the same thing by a different name in order to tax it more. After all, life is a planetary phenomenon, albeit not as transient as a hurricane or as inconsequential as a continental tectonic plate. Yet even the single example of life we know has the property of being transplantable (to another “lifeless” planet). Or at least we think so.
How can this be so? As I suggested in my parable of the three planets, we already know that the chemical process known as
life is sufficiently powerful to transport itself from Earth to the Moon, and there's a good chance that we've already sent bacteria as passengers on our spacecraft to live permanently on Marsâand maybe far beyond, whether on Titan thanks to Huygens or via Voyager to who knows where. But this kind of transplantation of microbial spores could have happened long before we began exploring space, and over greater distances. When Earth was being bombarded by asteroids early in its history, the outflow of material would have been nearly as great as the inflow, and it's not hard to imagine some of that material being life, ultimately to travel interstellar distances, embedded in dust particles or comets, until it found a new home on some other habitable planet. In that way, we can imagine planet Earth as a home or a cradle for life in the conventional sense. The idea is old and is called panspermia.
Panspermia, which means “seeds everywhere,” is an old idea that can be traced back to the Greece of Socrates. Svante Arrhenius, a Swedish chemist, revived the idea in the early twentieth century. Additional arguments were put forward by Fred Hoyle and Chandra Wickramasinghe in the 1970s, with comets as the delivery vehicle for spores, in order to protect them from radiation damage. (Hoyle and Wickramasinghe were also proponents of an eternal steady state Universe, with plenty of time for any spores to make their journey across our Galaxy and mix well among its stars. That kind of thinking made the Fermi paradox seem very appealing.) There is also a local version that postulates exchange within a planetary system, such as from Mars to Earth. The idea goes as follows: a large asteroid impacts young, wet Mars and ejects debris
into space and some into independent orbits around the Sun. Though such impacts resemble dramatic explosions (they melt and vaporize huge amounts of rock), many of the ejected rocks should remain entirely unheated and undisturbed. If Mars was alive, with microbes deep inside rocks, many microbial colonies should have survived inside large chunks of rock.
With timeâmillions of yearsâthe orbits of small rocks are perturbed by the changing gravitational pull of the planets. Often those orbits change dramatically and cross the orbits of large planets. Some of them end up on the surface of a neighbor planet as meteorites. There are many Martian meteorites on Earth; they are relatively easy to identify by their minerals and inclusions of small bubbles containing Martian atmosphere. This suggests that if life ever existed on Mars, it could have reached this planet too. Interestingly, it is far less likely to have gone the other way, as the predominant traffic flow is from the outer Solar System in. When orbits change, they tend to preferentially move toward the Sun.
Whether this has happened is simply an empirical question because the mechanism itself is quite plausible. We know that there are microbes durable enough to make the trip into space and endure the low temperatures and high radiation, even over the million years required for a typical “meteoritic” trip from Mars to Earth. Similar trips between stars, though possible, would take much longer.
1
Even if spores might survive billion-year journeys (no such spores are known today), the question remains if there has been enough time in the history of our Galaxy for such journeys to be completed yet. As noted in previous chapters, our Universe is young and the
components needed for life are even younger. Just as there has been barely enough time for life to have evolved at all, there is even less for spores to have dispersed through the volume of our big Galaxy. Even if panspermia is not the state of life today, it surely could be in its future.
Panspermia is a long-term phenomenon, however, and so the future of life, at least on our planet, may seem doomed to go on much as it has in the sense that evolution will continue operating on the same basic rules, with the same basic tools, such as DNA and RNA. But that scenario, I think, is far from the truth. In fact, we are living through, in the first years of the twenty-first century, a remarkable turning point in the history of life on Earth. For the first time in about 4 billion years a new species is
not
going to emerge from the set of processes that led to the diversity of life on this planet. Instead, one species is going to synthesize anotherâa life-form that is unique, but not in the way that a new dog breed or a genetically modified corn plant is made unique by some cosmetic differences with its progenitor. It will be new in terms of its unique biochemistry, a new life-form that has no place on Earth's tree of life, a new life-form at the root of a
new tree of life
.
I am describing the dawn of a new fieldâsynthetic biology. All Earth life has a striking unity of shared biochemistry, which is why we can use
E. coli,
fruit flies, and lab mice as proxies for our own biology in the laboratory. Much of the cellular machinery is the same. Through synthetic genomics (or, more generally, bioengineering) scientists use that unity to create diversity in form and function. Think of age-old breeding, or the current promise of fully designed microbial
genomes.
2
Synthetic biology, the way I refer to the field here, goes beyond synthetic genomics, both conceptually and in practice. It is no longer a matter of modifying a genome or even writing a new one, but of synthesizing biological systems that do not exist as such in nature and using this approach to understand life processes better.
3
This amounts to changing the basic biochemistry and that is why this new life-form cannot belong on Earth's tree of life.
Defined thus, synthetic biology research is closer to chemistry than to biology, while the opposite is true for genetic engineering.
4
(This led Pier Luigi Luisi to coin the term “chemical synthetic biology” in 2007.)
5
I see as one of its central concepts and objects of study the minimal artificial cellâa hypothetical chemical system enclosed in a vesicle and capable of life's main functions.
6
It is a cell because of the vesicle; it is “minimal” because it is stripped down to a bare minimum of function needed for self-sustained existence and evolution; it ought to be “artificial” because no one expects to discover such a primitive (and vulnerable) chemical system on Earth, where even the simplest microbes are highly sophisticated, complex cells and have occupied every conceivable space and niche. At the opposite end, a genetic engineer works with these same highly sophisticated forms, including plants and animals, and strives to modify them to perform in ways that go beyond their naturally endowed skills.
Synthetic biology brings the prospect that an alternative biochemistry is possible and may have developed independently on other planets (or on Earth). This prospect is remarkable in that it involves ordinary chemistry, as opposed
to discovering a new form of matter or a new force. Instead, the anticipated discovery lies in uncovering new basic rules (or “laws”) of nature. It is also remarkable because it allows us to ask the origins of life question in a way that may lead science to a breakthrough: What is the role of initial conditions in shaping the unity of Earth biochemistry? Does the diversity of planetary environments map onto a diversity of alternative biochemistries?
This prospect is significant because it pulls together the various threads woven throughout this book, in a synthesis that was hardly possible until very recently. The work in the lab has direct implications for the astronomical search for life on distant planets, and vice versa: these remote findings help hone the lab search for pathways to alternative biochemistries. To find if we are not alone in the Universe, we must understand life, and to understand life, we must learn how to build its chemistry.
When we try to grasp the challenge, the unity of Earth biochemistry can provide a helpful clue. Think of all chemistryâits millions of molecules and all the reactions that can take place among them under different conditionsâas a large space. Mathematically speaking, that is a multidimensional space that human brains can't visualize, but if we choose a few relevant parameters and reduce the many dimensions to two or three, we can see patterns that often resemble a geographical map with valleys and mountains. Scientists often call such spaces “landscapes.” The chemical landscape is shown in
Figure 12.1
.
Â
FIGURE 12.1
.
The chemical landscape shown here is a metaphor illustrating the space of all molecules and their chemical reactions, in terms of, for example, molecular reactivity. Surrounding the space are the entry points for assembling molecules (from atoms) under different environments. We call these “initial conditions.” Often an initial condition can occur in nature, as on early Earth; often it can only be achieved in a lab. One of the highest peaks in the landscape represents Earth's life biochemistry; a pathway from early Earth initial conditions leads to it. We don't know if other biochemistries exist, nor do we know if multiple pathways reach them or reach Earth's biochemistry (or its mirror handedness version: the second peak nearby).
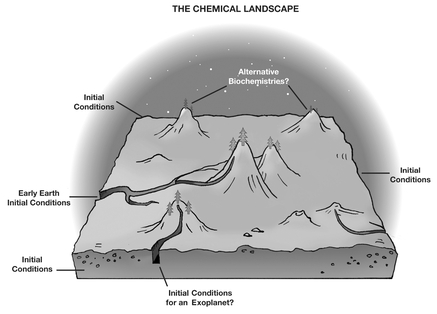
The most extraordinary feature of the chemical landscape is a high peakânarrow and well-definedâwhich encompasses all of Earth life biochemistry. The peak is high because life is a highly efficient chemical system today; evolution has raised its height through time. Its narrow base, on the other hand, illustrates how constrained in its chemical choices life is. It uses only twenty amino acids, only left-handed ones, only some proteins, only RNA and DNA, and so on, out of many millions of available choices in the vast chemical landscape. Are there any other tall peaks in the landscape? If so, which of them are alternative biochemistries (“other life”)? Are any of them accessible from plausible planetary environments?